Gain new perspectives for faster progress directly to your inbox.
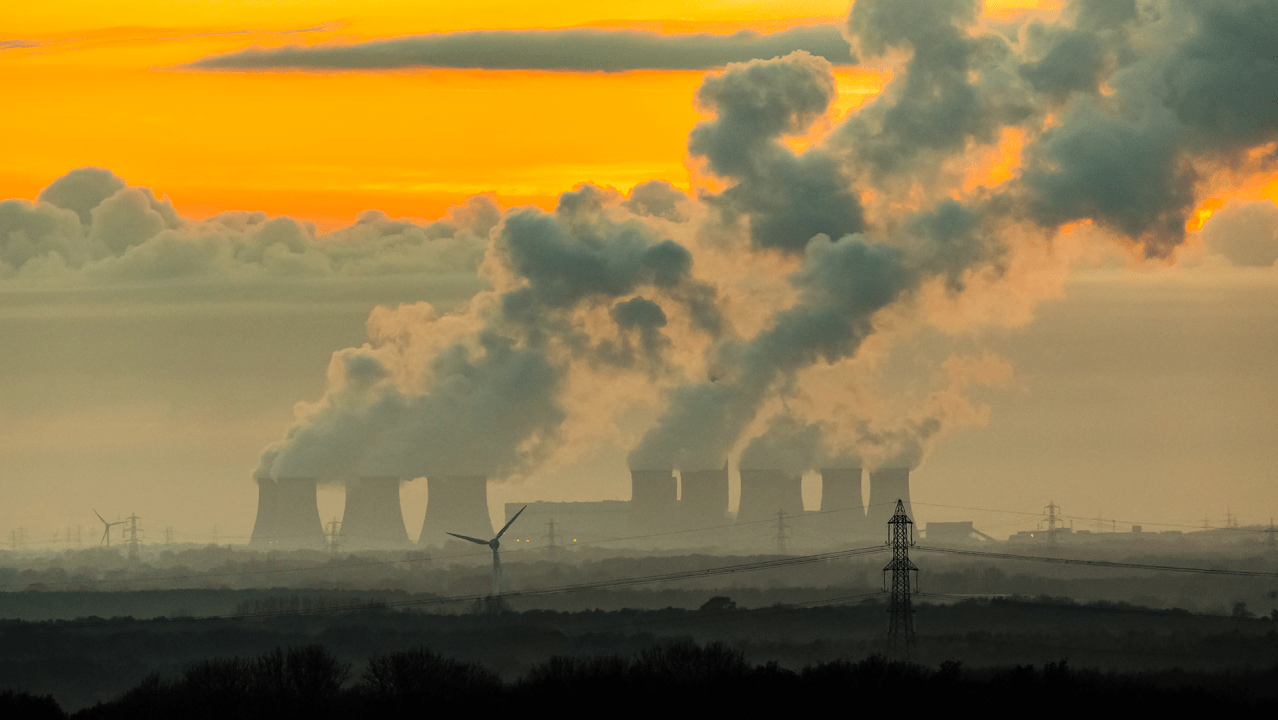
While human capabilities have only increased due to recent technological and medical advances, they have significantly contributed to the release of around 830 gigatons of CO2 into the atmosphere in just the last 30 years alone. The United Nations has pledged to “net zero” emissions by 2050, meaning that the amount of CO2 released into the atmosphere will also be removed. Achieving this goal will require the collaboration of scientists, policymakers, and industries worldwide.
In this journal manuscript on ChemRxiv, the CAS Content Collection was leveraged to showcase an analysis of how scientists and industrialists have used different approaches to restore carbon balance in the environment. The article includes a unique landscape view of the emerging topics, the latest trends in this area as well as the challenges currently faced. Read the full document here.
- Xiang Yu
CAS, a division of the American Chemical Society ,
- Carmen Otilia Catanescu CAS, a division of the American Chemical Society ,
- Robert Bird CAS, a division of the American Chemical Society ,
- Sriram Satagopan CAS, a division of the American Chemical Society ,
- Zachary J. Baum CAS, a division of the American Chemical Society ,
- Qiongqiong Angela Zhou CAS, a division of the American Chemical Society